Mass spectrometers, mass spectrometry, and climate change
From molecules to mountains, mass spectrometry zooms in on nature's secrets.
As an isotope geochemist, I spent most of my time working on large mass spectrometers. Since this technique and instrument are essential to our understanding of past climate, I thought I would give a brief intro to mass spectrometry and mass spectrometers.
Mass spectrometry…
Mass spectrometry (MS) is an analytical technique that identifies the amount and type of chemicals in a sample by measuring the mass-to-charge ratio and the abundance of gas-phase ions. In essence, it produces a spectral signature that represents the sample's unique composition.
The underlying principle of MS is ionizing chemical compounds to generate charged molecules or molecule fragments and then measuring their mass-to-charge ratios. These ions are generated in a source and are characterized based on their movement through an electromagnetic field, which can be influenced by their mass and charge. Thus, by analyzing these ion movements, we can deduce their mass-to-charge ratios and consequently the molecular composition of the sample.
The history of mass spectrometry traces back to the early 20th century. J.J. Thomson, who discovered the electron in 1897, performed the first mass spectrographic experiments in the early 1910s.
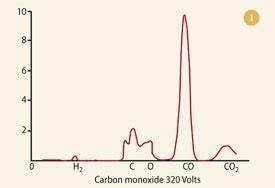
These experiments made use of magnetic and electric fields to analyze positive ions. By the 1920s, Arthur J. Dempster and F.W. Aston further refined the method, leading to the discovery and quantification of isotopes, which earned Aston the Nobel Prize in Chemistry in 1922.
Over the decades, various methods of ionization and analysis have been developed, allowing for the broad application of mass spectrometry in diverse fields. The 1980s and 1990s saw rapid advancements in the technique, particularly with the introduction of soft ionization techniques like matrix-assisted laser desorption/ionization (MALDI), electrospray ionization (ESI), and secondary ion mass spectrometry (SIMS). These allowed for the analysis of more complex molecules, such as proteins and polymers, without fragmenting them extensively as well as very small samples like single grains of zircon.
Today, mass spectrometry plays a crucial role in numerous areas, including earth science. Its ability to provide detailed and precise information about the composition of chemical samples makes it an indispensable tool in modern analytical chemistry.
Mass spectrometers…
Mass spectrometers have evolved, becoming increasingly sophisticated as researchers sought to analyze a broader range of compounds with greater precision. The various types of mass spectrometers are differentiated mainly by their ionization methods and mass analyzers, which have unique characteristics tailored for specific applications.
In the early days of mass spectrometry, the magnetic sector instrument was prominent, thanks to the pioneering work of J.J. Thomson, F.W. Aston, and others. These instruments, which use magnetic fields to bend the path of ions and separate them based on their mass-to-charge ratios, were crucial in the discovery and quantification of isotopes.
Time-of-flight (TOF) mass spectrometers emerged in the 1940s. Developed by William E. Stephens, TOF instruments measure the time ions take to travel a fixed distance. Because lighter ions travel faster than heavier ones, this method provides a means to determine the mass of the ions.
Quadrupole mass spectrometers made their appearance in the 1950s. These devices employ oscillating electric fields to filter ions based on their mass-to-charge ratios. Quadrupoles can act as mass filters, letting only ions of a specific mass-to-charge ratio reach the detector.
In the late 20th century, ion trap mass spectrometers became prominent. These instruments can trap ions in a three-dimensional space using radiofrequency electric fields, allowing for extended analysis and multiple stages of mass spectrometry in one device.
The advent of soft ionization techniques in the 1980s, such as matrix-assisted laser desorption/ionization (MALDI) and electrospray ionization (ESI), was a game-changer. These techniques allowed for the ionization of large and fragile molecules like proteins without breaking them apart, making them especially valuable in the field of proteomics.
Secondary Ion Mass Spectrometry (SIMS) is another pivotal technique in the mass spectrometry world and the one that I had the opportunity to work on during my time at UCLA. SIMS operates on the principle of sputtering, where a primary ion beam is directed at a sample, resulting in the ejection of atoms or small clusters from the surface. Some of these ejected particles are ionized, and these secondary ions are then analyzed by the mass spectrometer. The method is highly surface-sensitive, making it especially adept at analyzing the composition of thin films, interfaces, and small surfaces such as a grain of zircon for geochronology.
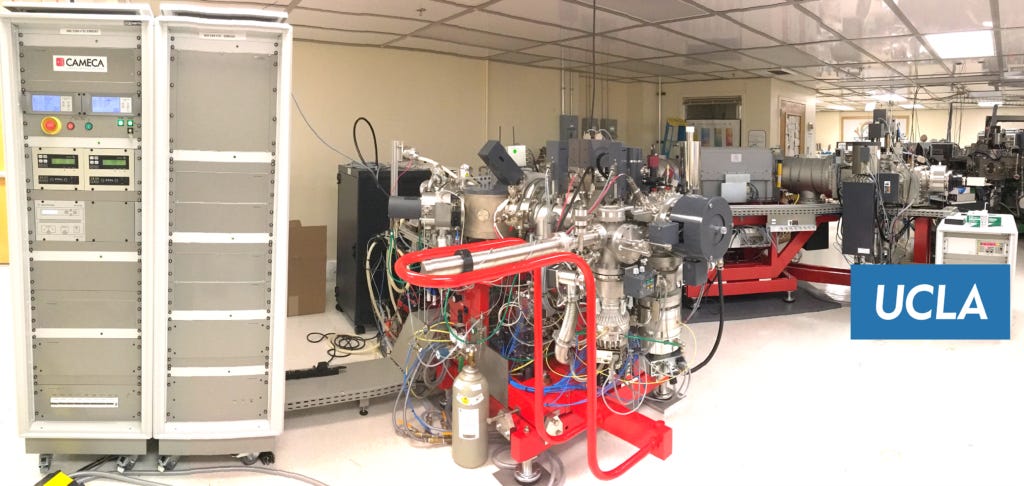
Today's mass spectrometry landscape is diverse, with each type of instrument having specific strengths tailored to various analytical needs. The evolution of these instruments over the past century reflects the field's dynamic nature, continuously adapting to address new challenges and broaden the scope of its applications.
Accuracy of mass spectrometers…
Mass spectrometry, since its inception in the early 20th century, has witnessed tremendous advancements, leading to remarkable improvements in the accuracy of measurements.
In the early days, mass spectrometers, primarily magnetic sector types, offered a relatively crude but revolutionary means of distinguishing ions based on their mass-to-charge ratios. Influences like magnetic field inhomogeneities and temperature fluctuations impacted the paths of ions and thus the accuracy of measurements.
By the mid-20th century, advancements in technology, including better vacuum systems and refined detector designs, enhanced the capabilities of mass spectrometers.
But it was perhaps the advent of quadrupole mass analyzers in the 1950s and 1960s that marked a significant leap in the accuracy and reliability of mass spectrometers. Quadrupoles could isolate specific ion masses with high precision, leading to better specificity in measurements and reducing the influence of overlapping signals.
The late 20th century saw further innovations, such as the ion trap and Orbitrap mass analyzers, which offered incredibly high resolution and, subsequently, improved accuracy. Coupled with the development of soft ionization techniques, mass spectrometers became equipped to accurately measure more samples.
In parallel with these hardware advancements, software and data processing techniques have also evolved, enabling more accurate interpretation of complex mass spectra, error correction, and calibration methodologies.
Today's state-of-the-art mass spectrometers are capable of measuring the mass of ions with extraordinary accuracy, often to the fourth or fifth decimal place, and they can reliably detect minute differences in isotopic abundances or subtle modifications in molecular structures.
Precision of mass spectrometers…
The precision of measurements in mass spectrometers is closely tied to the statistical nature of ion detection. Given that ions are detected and counted individually, the process inherently introduces statistical noise, which can limit the overall precision of the measurements. This statistical noise is often governed by counting statistics, particularly when considering different detectors used in mass spectrometers.
Different detectors, such as electron multipliers, Faraday cups, and ion-to-photon detectors, each come with their nuances in terms of efficiency, dynamic range, and noise characteristics. For instance, electron multipliers amplify the signal from detected ions by triggering a cascade of electron emissions. While this can significantly boost sensitivity, the amplification process can introduce noise, especially at lower ion counts. This is because, at very low ion counts, the variance in the number of ions detected can be comparable to the mean number of ions, leading to significant relative fluctuations in the signal.
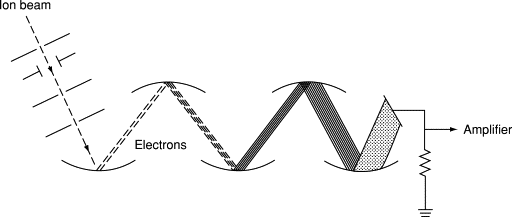
Faraday cups, on the other hand, measure the current produced by ion impacts directly. While they can be more stable and linear over a wide dynamic range, they might not be as sensitive as electron multipliers for very low ion counts. Thus, in situations where only a few ions are being detected, the inherent statistical fluctuations in the ion count (following Poisson statistics) can become a significant factor limiting precision
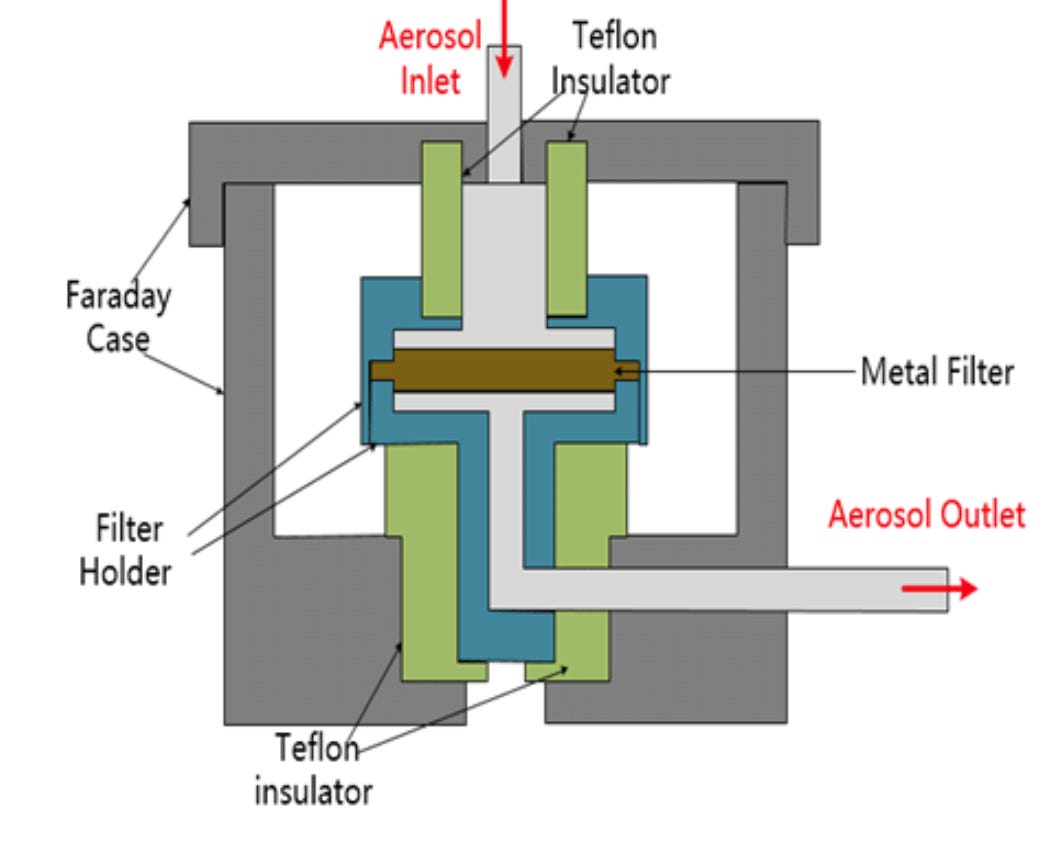
The noise introduced by the statistical nature of ion counting can be particularly problematic when trying to determine low-abundance isotopes or trace compounds in a sample. In such cases, the signal-to-noise ratio can be a limiting factor, and the counting statistics can pose challenges for distinguishing genuine signals from background noise.
In practice, the precision of mass spectrometric measurements is not soly determined by the detector's counting statistics. Other factors, such as the stability of the ion source, the resolution of the mass analyzer, and electronic noise, also play roles. However, the inherent statistical nature of ion detection means that there will always be a fundamental limit to precision, especially when working with low ion counts. It's a constant challenge for researchers and instrument designers to find ways to mitigate these limitations and extract meaningful data from the noise.
Standards in mass spectrometry…
Mass spectrometry, like many analytical techniques, often requires the use of standards to ensure both precision and accuracy in measurements. The inclusion of standards addresses various inherent challenges and improves the reliability of mass spectrometric data.
Standards, which are compounds with known concentrations and compositions, serve as references. They can be introduced into the sample or run separately to calibrate the instrument or validate the results. There are several types of standards used in mass spectrometry, including internal standards (added directly to the sample) and external standards (analyzed separately from the sample).
Precision is the measure of how closely repeated measurements agree with each other. When standards are employed, particularly internal standards, they help account for variations that can occur during sample preparation, ionization, and detection. If, for example, the ionization efficiency drops during an analysis, the response from both the standard and the analyte will be affected similarly. By comparing the analyte response to the standard response, this variability can be corrected, leading to more consistent (precise) results across multiple measurements.
Accuracy reflects how close a measurement is to the true value. The use of calibration curves, derived from analyzing multiple concentrations of a standard, helps in determining the actual concentration of an analyte in a sample. If the mass spectrometer tends to drift over time or if there are systematic errors in the measurement process, comparing results to a known standard can help identify and correct these discrepancies, ensuring that the measurements are accurate.
Moreover, for isotope ratio mass spectrometry, which measures the ratios of isotopes in a sample, the use of standards is crucial. Any deviation from the expected isotopic ratio in the standard can be used to correct measurements of the sample, ensuring accuracy.
The choice of an appropriate standard is critical. Ideally, the standard should behave similarly to the analyte in terms of ionization efficiency and chemical properties. If the standard and analyte respond differently to changes in the instrument or experimental conditions, the corrections might not be valid.
Mass spectrometry, mass spectrometers, and climate change…
Keep reading with a 7-day free trial
Subscribe to Irrational Fear to keep reading this post and get 7 days of free access to the full post archives.